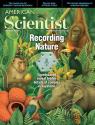
This Article From Issue
July-August 2025
Volume 113, Number 4
Page 195
Origins of Consciousness
To the Editors:
In Consciousness: The Road to Reductionism, Alan J. McComas presented an interesting history of research on neural activity and its relationship to consciousness. The key issue is whether a reductionist view of the brain as pure biology—which for some extends to questioning whether humans possess free will—is better than earlier syntheses in which some nonbiological entity (such as a soul or psychic energy) is the source of consciousness. Although McComas often uses qualifying phrases such as “interpreted by some,” I suspect that he favors the reductionist viewpoint by the absence of arguments as to why it might not be right.
I’m not supporting the idea of a nonbiological entity, but I would suggest that biology may still be able to provide an explanation of why humans overwhelmingly think that they possess the ability to make decisions that influence future events. I accept that the deterministic alternative may be true, but if it is not, then the adoption of a fatalistic attitude may lead to suboptimal decision-making.
It’s easy to see why a random mutation in the brain that led to decision-making would provide an evolutionary advantage. It’s harder to see why a mutation that led one to believe in free will when it didn’t really exist would be advantageous. I’m unlikely to give up on free will until a good biological explanation is provided of how innovation and creativity arise without intent.
Stephen L. Brown
Alameda, CA
To the Editors:
Alan J. McComas has laid out with great clarity the reductionist’s perspective on consciousness. That clarity permits one to examine the perspective’s strengths considering that consciousness remains undefined and there is a more parsimonious perspective for explaining what is more accurately referred to as “behaving consciously.”
By concentrating on the brain to hopefully reveal the neural structures that explain consciousness, what is overlooked is the human organism that is said to be conscious. It is the living organism that reports that it is conscious of something—that is, there is no consciousness in the abstract.
The cumbersome model composed of sensation, perception, memory, and information processing ignores the living organism interacting with the environment. The organism responding to the social and physical environment produces effects that research has repeatedly shown can result in new behavior as well as increasingly complex antecedent–behavior–environment relationships.
When the response-environment contingencies are of a particular character, a person acquires responses that we call conscious. Rather than searching for consciousness in the brain, we can observe the characteristics of behaviors that we define as conscious. We can then examine the environmental circumstances that encourage the person to behave consciously and discover the consequences to strengthen and maintain conscious behaviors.
Continued neurological research is to be valued in its own right. But the search for how we become conscious requires investigation into environmental contingencies that lead to learning such responses. Neural correlates are also present, but they are not a sufficient explanation on their own.
Robert Jensen
Sacramento, CA
Dr. McComas responds:
I agree with several of the points Dr. Brown and Dr. Jensen raise. On one point, however, I will not budge––consciousness is entirely a product of the brain (or its counterpart in invertebrate nervous systems). Consciousness has functional value, as would be expected of any life feature that, during evolution, has become ever more prominent.
In the case of free will, it is true that we can nearly always find a reason for an action or thought, namely something we have seen, heard, read about, or imagined. On the other hand, consciousness, though a product of neurons, influences their further activity. As an example, conscious images of possible actions and their consequences will determine the next phase of neural activity (an apparent choice based on the pleasure or discomfort associated with the imagery). More than that, consciousness provides us with memory, justifies our actions, and gives us the indispensable illusion that we are in charge of ourselves.
I agree with Dr. Jensen that consciousness is a property that the nervous system learns following receipt of information from the internal and external environments. In other words, consciousness is acquired rather than an innate feature of the fetal and neonatal nervous systems. Dr. Jensen’s point that “neural correlates may be necessary but they are not sufficient” is the “hard” problem of consciousness—the mechanism whereby impulse activity in neurons is transformed into our thoughts and sensations. Not only have we neuroscientists not solved this problem, but it is impossible to conceive of the basis for a solution. Though that response may be seen as a cop-out, I wonder whether, given the underlying neural activity, consciousness is a natural phenomenon––making it a fact rather than a problem.
Finally, an apology for an error that somehow crept into the text of my article. Subjects in the Los Angeles concept cell experiments viewed an image on the computer screen for a second rather than a minute.
Quantity and Quality
To the Editors:
Publishing is an integral part of the job and the joy of doing science. I still recall the thrill when invited to publish findings from my doctoral dissertation in Scientific American, which I had read since I was a teenager. Combining published Dryopithecine tooth dimensions and molecular data, I hypothesized shifting the ape-human divergence from 14 to 15 million years ago to 6 to 8 million years ago. Three decades later, my lab analyzed MRI scans of fossil femoral fragments and found internal bone structure diagnostic of upright posture at 6 million years ago (published in Science, with support from a Sigma Xi GIAR). My personal experience is reinforced by much better-known examples, such as the discovery by Barry Marshall and Robin Warren that the primary cause of peptic ulcers is infection by Helicobacter pylori, and the invention of polymerase chain reactions by Kary Mullis. Both Nobel Prize–level breakthroughs were preceded by very few publications by the investigators.
Against this background I offer two reflections on the article The Case for Quantity in Science Publishing by David B. Allison and Brian B. Boutwell. First, the primary objective of scientific research is not publication but rather solving problems. Second, the quantity of publications is not a reliable indicator of the importance of the problem or of progress toward solving it.
Robert B. Eckhardt
State College, PA
Dr. Allison and Dr. Boutwell respond:
Dr. Eckhardt does not wish for quantity of publications to serve as the primary objective of science, and neither do we. We concur that continually proposing and empirically scrutinizing novel solutions to problems might rightly be seen as our primary objective as scientists. Yet the practical utility we glean from science does nothing to diminish the esoteric beauty and wonder we often experience when the last puzzle pieces on some topic fall into place.
Dr. Eckhardt argues that science is about solving problems and that only counting the number of publications would be a poor barometer for gauging success on that front, and on that point we agree. But if we think problem-solving is a process of discarding ineffective solutions in favor of better ones, it seems that the following excerpt from our essay should also be a point of agreement: “Quantity makes it easier for investigators to engage in competition and probe for weaknesses in an idea.”
We sought to challenge the view that quantity and quality are diametrically opposed, and that encouraging quality must involve discouraging quantity. One will not find any objection from us to striving to improve the quality of scientific research. What readers will find in our essay are reasons why better quality will not emerge from strategies simply designed to discourage quantity.
American Scientist Comments and Discussion
To discuss our articles or comment on them, please share them and tag American Scientist on social media platforms. Here are links to our profiles on Twitter, Facebook, and LinkedIn.
If we re-share your post, we will moderate comments/discussion following our comments policy.