
This Article From Issue
September-October 2005
Volume 93, Number 5
Page 462
DOI: 10.1511/2005.55.462
Conflict in the Cosmos: Fred Hoyle's Life in Science. Simon Mitton. xviii + 401 pp. Joseph Henry Press, 2005. $27.95.
Conflict in the Cosmos is a warm appreciation and cogent assessment of the scientific life of the British astrophysicist Fred Hoyle. Hoyle, who died in 2000, was one of the most capable and controversial theorists of the 20th century, contributing provocatively to a wide range of problem areas, from stellar structure and the origin and evolution of the chemical elements to the large-scale structure and history of the universe.
The author, astronomer Simon Mitton, is at his best when introducing and then explaining in simple language the scientific underpinnings of Hoyle's theories. He also clearly recounts Hoyle's life, training and career, helping the reader to better appreciate the world of Cambridge academics.
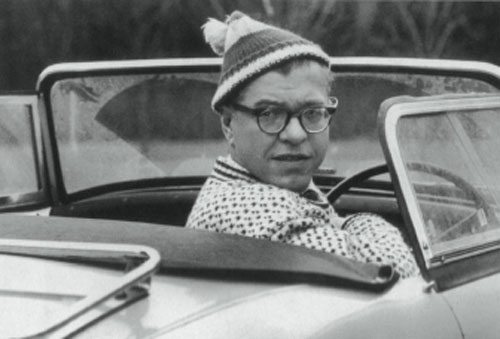
From Conflict in the Cosmos.
Born in Yorkshire in 1915, the son of a woolens merchant and a former schoolteacher, Fred Hoyle was sent to a local "Dame" school (a private one-room school with a single teacher) and then to elementary schools, where he distinguished himself first by his truancy. His talents emerged as he was slowly attracted to science, and then he rapidly went straight to the top of whatever academic ladder was available to him. In 1933 he entered Emmanuel College at the University of Cambridge, and his life was centered there for more than 39 years, with the significant exception of his wartime service, which involved working on the design of radar systems and taking lengthy research trips to America.
Hoyle rose from a mathematics lectureship to assume the Plumian Professorship of Astronomy and Experimental Philosophy in 1957, holding that position until his heated and painful resignation in 1972, when he also terminated his relations with the Institute of Theoretical Astronomy, which he had founded and directed for six years. During this span of years, Hoyle had become an international figure in science and enjoyed a great deal of public visibility through his radio broadcasts and his popular science and science fiction writings. He had also become a power broker in the British scientific establishment. Yet he remained very much an outsider, accumulated passionate enemies and espoused unpopular theories.
As a result, much has been written about Hoyle; his autobiography is extensive, and he and his colleagues wrote numerous essays covering aspects of his scientific life. The book under review here is the first significant biographical effort by a witness who was not a colleague.
Simon Mitton is well known as the science director at Cambridge University Press and as author and coauthor of many popular works on astronomy. His postgraduate training was at Cambridge, in Martin Ryle's radio astronomy group, and then he became a member of the institute Hoyle had founded, taking an academic management post there in the wake of Hoyle's resignation and departure. Mitton is therefore in a rather good position to provide an institutional as well as intellectual profile of Hoyle's years at Cambridge and in the British scientific establishment.
The book is solid but has the usual odd blunders and biases. Hoyle was surely not 15 in 1915 (as Mitton states on page 23) and was also not the eponymous source of the popular phrase "according to Hoyle," as Mitton seems to imply on page xvi (the real source was Edmond Hoyle, the author of a popular 18th-century book on the game of whist). Slips such as these are minor, although a bit too plentiful.
At the outset, I was concerned that Mitton might regale the reader with accounts of how the world of astronomy had misunderstood or mistreated Fred Hoyle or even conspired to thwart his spectacular talents, energy and drive. And indeed, some of Mitton's claims early in the book, particularly that "Fred Hoyle completely transformed British astronomy in the quarter century beginning about 1950," were quite worrisome. But happily, my fears were not realized. Mitton, although his writing is filled with deep respect, admiration and even awe for Hoyle's intellectual capabilities and attainments, is also almost always refreshingly balanced and probing in his analysis of Hoyle's fate, recognizing that it was often as much the result of Hoyle's own actions as of those of his competitors. Specifically, Mitton's treatment of Hoyle's resignation from Cambridge is most illuminating. Hoyle became enraged, charging conspiracy and collusion, when he believed he was not properly consulted in the deliberation to designate the head of a new combined organization merging his institute with Cambridge Observatories. Mitton shows, clinically, how such decisions were made at Cambridge and how Hoyle indeed deluded himself.
Mitton makes much of Hoyle's famous contempt for authority, a flair he traces at least as far back as Hoyle's early childhood and the influence of his father. Mitton also well identifies the equally difficult personalities with whom Hoyle clashed, or in one famous case, allied himself. Certainly Martin Ryle stands out as Hoyle's chief antagonist, and a large portion of the book is devoted to exploring the profound depths of their troubles. Those took on their greatest significance when Ryle and a group of radio astronomers he led claimed to have established evidence that the universe was evolving—not static, as Hoyle, Hermann Bondi and Thomas Gold had envisioned in their "steady state" model.
The most fascinating, and saddening, of Hoyle's allies was Ray Lyttleton, a brilliant mind who was unable to manage honest differences of opinion or of fact. Mitton's portrayal of Lyttleton would be almost impossible to believe unless one knew that the man's remarkably iconoclastic behavior was already well established by the time he allied with Hoyle. Indeed, if Mitton had referred to contemporary historical literature, something he does very sparingly, it would have helped to clarify that Lyttleton's reputation was well established before he teamed up with Hoyle.
Mitton knows full well that Hoyle rarely cited anything other than the efforts of contemporary workers and slighted earlier literature. This is obviously the case in Hoyle's 1994 autobiography, Home Is Where the Wind Blows (University Science Books), an intensely thrilling yet oddly rambling effort. To place Hoyle's work in fuller historical context, Mitton should have taken more care to probe the histories of concept formation beyond the citations in Hoyle's own papers. An especially important case in point is the erroneous claim that Hoyle "was the first to replace the iron Sun with one overwhelmingly dominated by hydrogen and helium." At least three sources in the recent historical literature trace this advance to other researchers (such as Bengt Strömgren and Thomas Cowling) working a decade before Hoyle.
Another concern about many of Mitton's sweeping statements is the lack of adequate citation or documentation. Although he generously cites personal sources, interviews, letters, e-mails, scientific articles and books, there are some very important passages where there are very few citations. At times I was unsure whether the author was voicing a personal opinion from experience or from his extensive contacts within Hoyle's circles of students, colleagues and friends, or whether, indeed, he was voicing the collective opinion from uncited reviews or even secondary historical works. Part of the problem surely lies in the complexity of Hoyle's academic life, which had many intertwined and contingent elements: Cambridge politics; college, laboratory and institutional rivalries; and national policy formation. Unfortunately, Mitton's lack of pointed referencing makes it impossible to determine exactly who held what opinion at what point in time. It is difficult, in other words, to evaluate whether something is the author's opinion or an actual reflection of the sides taken in these policy debates.
Mitton is to be congratulated for providing insight into a particularly fascinating character. But this biography is not definitive. It is certainly not the case that no one will want to delve into the life of Fred Hoyle again—there is much left to do. After all, Hoyle acted as a provocateur—probing and, yes, disturbing the professional communities he touched. No historian who wants to understand how communities of science define and structure themselves, intellectually as well as institutionally, can ignore the importance of watching how the organism reacts. A good start has been made right here.
American Scientist Comments and Discussion
To discuss our articles or comment on them, please share them and tag American Scientist on social media platforms. Here are links to our profiles on Twitter, Facebook, and LinkedIn.
If we re-share your post, we will moderate comments/discussion following our comments policy.