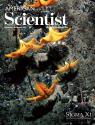
This Article From Issue
November-December 2010
Volume 98, Number 6
Page 509
DOI: 10.1511/2010.87.509
GLIMPSES OF CREATURES IN THEIR PHYSICAL WORLDS. Steven Vogel. xiv + 302 pp. Princeton University Press, 2009. $35.
Back when clocks had gears, springs and hands, manufacturers issued special pocket watches to their salesmen. These clever advertising gimmicks had the usual crystal on the front so that one could tell the time, but in addition they had a crystal on the back so that customers could watch the gears move, count the oscillations of the escapement and marvel at how much machinery was packed into such a small case. Steven Vogel, a biology professor at Duke University, is clearly privy to the watchmakers’ insight that we humans are fascinated by how things work. His latest book, Glimpses of Creatures in Their Physical Worlds, is the literary equivalent of a glass-backed watch—an invitation to explore the workings of nature through the perspective of biomechanics.
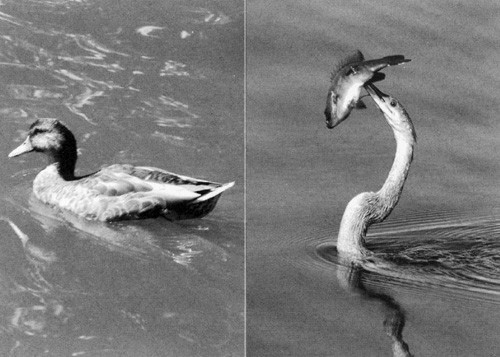
From Glimpses of Creatures in Their Physical Worlds.
Often described as the application of physics and engineering to the study of organisms, biomechanics is a burgeoning branch of physiology. The field proudly traces its roots back to Galileo, who in his final book, Discourses and Mathematical Demonstrations Relating to Two New Sciences (1638), noted that the leg bones of large mammals—rhinoceroses and elephants, for instance—are much thicker relative to their length than are the gracile leg bones of smaller mammals such as cats and rabbits. He reasoned that if organisms and their skeletons maintained the same shape across size, weight would increase with volume and therefore with the cube of an organism’s height, whereas the ability of a bone to resist weight would increase with its cross-sectional area, and therefore with only the square of height. The increasing ratio of weight to area would thus place bones of large animals at risk of breaking. To avoid that risk, the shape of the legs had to change.
I was introduced to this elegant idea in 1973 when I took an undergraduate course in biomechanics from Vogel and his colleague Stephen A. Wainwright. Despite having an ancient heritage, biomechanics was at that time a newly emerging field, and Vogel and Wainwright were two of its joyfully evangelical proponents. Along with R. McNeill Alexander at the University of Leeds in England, they produced a series of books with the avowed intention of attracting interest in their mechanical perspective on biology. Now, some 37 years later, biomechanics is an established (albeit small and quirky) discipline, with practitioners around the globe.
Vogel’s contributions to biomechanics have had two admirable objectives. In Life in Moving Fluids (1981), Life’s Devices (1988), Vital Circuits (1992), Prime Mover (2001) and Comparative Biomechanics (2003), his goal is to explain the mechanics of biology to a general audience. If you want to know how fish swim, fleas jump and bats fly, or why hardening of your arteries is a bad thing, then dip into these sources; you will come away both informed and amused. Vogel is a gifted teacher who has the knack of explaining complex processes in a fashion that imparts both knowledge and insight. He also has the soul of a punster and delights in spicing his prose with unexpected wordplay. Although the subject matter of these books is nontraditional, they adhere to the traditional perspective that the task of the biologist is to observe nature and explain what is seen. For example, it has been observed that animals need to inspire oxygen and dispose of carbon dioxide, whereas plants must do the reverse. Vogel notes this and provides an explanation of the contrasting mechanics involved: Reynolds numbers, Péclet numbers, Bernoulli’s equation—the whole nine yards. Terrestrial organisms must support themselves against the pull of gravity, and Vogel tells us how wood, bone, and insect cuticle manage this task in different ways. When it is cold, how do mammals, birds and fish such as tuna stay warm? When it is hot, how do dogs stay cool? Vogel has the answers.
But one can tell that Vogel, while hewing to the traditional perspective, has a hankering to buck it. All too often, biologists observe only what they are prepared to see. Vogel’s second objective is therefore to expand their perspectives by conjuring up and carefully analyzing systems that might be. As he puts it, “recognizing a possibility can provide a sieve, a search image, or a hypothesis.” For example, dogs don’t sweat as humans do. Instead, they pant, evaporating water from their respiratory tracts and expelling the resulting warm, moist air with each breath. But panting requires the repeated contraction of chest muscles, which adds to the heat the animal desires to lose. Could there be a better way? Engineers have devised “heat pipes” in which the evaporation of fluid itself provides the pumping action necessary to transport heat, no muscles required. Could a heat pipe work in animals?
To find out, read Glimpses of Creatures in Their Physical Worlds. Here, as in Cats’ Paws and Catapults (1998), Vogel takes a decidedly nontraditional look at biology, unleashing his talent for unbridled speculation. The 12 chapters of Glimpses, which began life as a series of essays in the Journal of Biosciences, have been revised and updated. They cover topics that range from the ballistics of seeds (plants use both catapults and cannons to launch their propagules) to the breathing apparatus of diving spiders (tiny hairs on the body take advantage of surface tension to maintain an airspace into which oxygen can flow), with stops along the way to explore the efficiency of man-made and natural pumps, the twist-to-bend ratios of daffodils in the breeze, and the physics of cow tipping. The following two paragraphs from the chapter on “Making and Maintaining Liquid Water” will give you the flavor of the discussion:
Ice may be the most widespread toxic substance afflicting life. Not that one can’t imagine positive roles for it. Even at constant temperature melting ice absorbs energy, so it could provide cooling elsewhere in a system—thus we cool beverages, freeze ice cream, and so forth. It can provide an osmotically inert and minimally volatile store of liquefiable water. Fractional crystallization as it forms can concentrate desirable solutes. Its lower-than-liquid density suits it as a flotation device. Adjusting the ratio of liquid to solid water in a system at the freezing point can stabilize temperature, permitting a kind of 0°C homeothermy. But as far as I know, all of these possibilities remain biologically hypothetical.
One can, though, point out a few instances where ice finds use. Ice has a low thermal conductivity so it sometimes provides insulation, especially in the flocculent form of snow, a cheap and disposable (but not easily portable) augmentation for fur or feathers. Water’s high heat of fusion means that relative to deposited mass, frost formation will buffer a nighttime drop in plant temperature from radiation to the sky better than will dew. Just as melting ice absorbs energy, freezing water releases it. So a slight temperature increase will accompany ice formation within organisms.
By my count, there are eight hypothesized biological uses for ice in those two paragraphs, five of them totally unexplored—perhaps an entire career’s worth of viable research topics. If what you desire in a readable science book is food for thought, Glimpses of Creatures in Their Physical Worlds provides a feast. Biologists, engineers and physicists—indeed, anyone with curiosity about the natural world—will revel in this smorgasbord of biomechanical ideas.
Mark Denny is John B. and Jean DeNault Professor in Marine Sciences Biomechanics at Hopkins Marine Station of Stanford University. His research centers on the mechanical design of intertidal organisms.
American Scientist Comments and Discussion
To discuss our articles or comment on them, please share them and tag American Scientist on social media platforms. Here are links to our profiles on Twitter, Facebook, and LinkedIn.
If we re-share your post, we will moderate comments/discussion following our comments policy.