
This Article From Issue
May-June 1998
Volume 86, Number 3
DOI: 10.1511/1998.25.0
Believing as they did that the epidemics were somehow related to the poor physical state of the city, Council members had already turned to the fundamental tasks of maintenance. In January 1794, they devised a long-term plan for "better cleansing the streets."
"Beyond Therapeutics." Michael McMahon
A Melancholy Scene of Devastation: The Public Response to the 1793 Philadelphia Yellow Fever Epidemic
J. Worth Estes and Billy G. Smith, eds.
Science History Publications/USA. $35.95
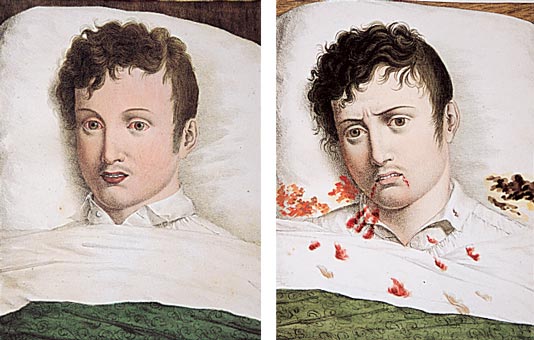
From A Melancholy Scene of Devastation.
A circular for consumptives distributed circa 1908 by the Illinois State Board of Health began, "FOLLOW THE GOLDEN RULE: Do unto others as you would that they should do unto you," and was followed by more prosaic injunctions such as "DON'T EVER SPIT ON ANY FLOOR, BE HOPEFUL AND CHEERFUL, KEEP THE WINDOW OPEN." In like fashion, the Modern Health Crusade modeled the war against tuberculosis on the Christians' crusades against the infidels; as one young crusader observed in a prize-winning essay, "The germs are the Turks."
The Gospel of Germs: Men, Women and the Microbe in American Life
Nancy Tomes
Harvard University Press. $29.95
The epizootic brought with it different degrees of fear. A man who trapped a stray cat at a trailer park in Edinburg (Texas) took it upon himself to kill the animal. He did this by squeezing its head—to suffocate it. The cat, understandably, fought for survival and scratched and bit him badly. This made the man angry, and he put the animal back in its cage and ran the whole thing over with a tractor. He then went to the doctor to get his wounds treated. The physician recommended testing what was left of the cat. It was positive.
Mad Dogs: The New Rabies Plague
Don Finley
Texas A&M Press. $14.95 (paper)
American Scientist Comments and Discussion
To discuss our articles or comment on them, please share them and tag American Scientist on social media platforms. Here are links to our profiles on Twitter, Facebook, and LinkedIn.
If we re-share your post, we will moderate comments/discussion following our comments policy.